3 Article 1
Assessing the validity of a calcifying oral biofilm model as a suitable proxy for dental calculus
3.1 Introduction
Dental calculus is becoming an increasingly popular substance for exploring health and diet in past populations (Warinner et al., 2015). During life, dental plaque undergoes periodic mineralisation, trapping biomolecules and microfossils that are embedded within the dental plaque biofilm in the newly-formed dental calculus. This process is repeated as new plaque is deposited and subsequently mineralises, resulting in a layered structure representing a temporal record of biofilm growth and development (Warinner et al., 2014). The calculus serves as a protective casing for the entrapped biomolecules and microfossils, preserving them for thousands of years after death and burial (Fellows Yates et al., 2021). Studies using archaeological dental calculus span a wide range of topics in different regions and time periods. These include characterisation of the oral microbiome and its evolution in past populations (Adler et al., 2013; Fellows Yates et al., 2021; Kazarina et al., 2021; Velsko et al., 2019; Warinner et al., 2014), as well as extraction of microbotanical remains (Hardy et al., 2009; Henry & Piperno, 2008; Ma et al., 2022; Mickleburgh & Pagán-Jiménez, 2012) and other residues to infer dietary patterns and nicotine use (Bartholdy et al., 2023; Buckley et al., 2014; Eerkens et al., 2018; Hendy et al., 2018; Velsko, Overmyer, et al., 2017). Dental calculus has already provided a unique and valuable insight into the past, but the exact mechanism of the incorporation, retention, and preservation of microfossils and biomolecules exogenous to the microbial biofilm is largely unknown; even the process of plaque mineralisation is not fully understood (Jin & Yip, 2002; Omelon et al., 2013). This means that there may be hidden biases affecting our interpretations of dietary/activity patterns extrapolated from ancient dental calculus. These biases have been explored archaeologically (Fagernäs et al., 2022; Tromp et al., 2017) as well as in contemporary humans (Leonard et al., 2015) and non-human primates (Power et al., 2015), but not experimentally.
Dental plaque is an oral biofilm and is part of the normal state of the oral cavity. However, when left unchecked, plaque can lead to infections, such as dental caries and periodontitis, and/or mineralisation (Marsh, 2006). The dental plaque biofilm grows in a well-characterized manner before mineralisation, in a process that repeats regularly to build up dental calculus. Shortly after teeth are cleaned (whether mechanically or otherwise), salivary components adsorb to the crown or root and form the acquired dental pellicle. The pellicle provides a viable surface for bacteria to attach, especially early-coloniser species within the genera Streptococcus and Actinomyces (Marsh, 2006). Once the tooth surface has been populated by specialists in surface-attachment, other species of bacteria can attach to the adherent cells, increasing the biofilm density and diversity. The bacterial species secrete polysaccharides, proteins, lipids, and nucleic acids, into their immediate environment to form a matrix that provides structural support, nutrition, and allows for environmental niche partitioning (Flemming et al., 2016).
Biofilms can become susceptible to calcification under certain microenvironmental conditions, including an increased concentration of salts and a decrease in statherin and proline-rich proteins in saliva, rises in local plaque pH, and increased hydrolysis of urea (White, 1997; Wong et al., 2002). These conditions can cause increased precipitation and decreased dissolution of calcium phosphate salts within saliva and the plaque biofilm. The resulting supersaturation of calcium phosphate salts is the main driver of biofilm mineralisation (Jin & Yip, 2002). The primary minerals in dental calculus are hydroxyapatite, octacalcium phosphate, whitlockite, and brushite. During initial mineralisation the main mineral component is brushite, which shifts to hydroxyapatite in more mature dental calculus (Hayashizaki et al., 2008; Jin & Yip, 2002). The exact elemental composition of dental calculus varies among individuals due to various factors, including diet (Hayashizaki et al., 2008; Ji et al., 2000).
Dental plaque can also be grown in vitro, and these oral biofilm models are commonly used in dental research to assess the efficacy of certain treatments on dental pathogens (Exterkate et al., 2010; Filoche et al., 2007) without the ethical issues of inducing plaque accumulation in study participants and the complexity of access and sampling in humans or animals. Oral biofilm models are often short-term models grown over a few days, but longer term models also exist (up to six weeks) which are used to develop mature plaque or dental calculus (Middleton, 1965; Sissons et al., 1991; Velsko & Shaddox, 2018; Wong et al., 2002). A well-known limitation of biofilm models is the difficulty in capturing the diversity and complexity of bacterial communities and metabolic dependencies, micro-environments, nutrient availability, and host immune-responses in the natural oral biome (Bjarnsholt et al., 2013; Edlund et al., 2018; Velsko, Cruz-Almeida, et al., 2017; Velsko & Shaddox, 2018). These limitations can be overcome by complex experimental setups, but at the cost of lower throughput and increased requirements for laboratory facilities.
Despite the limitations, oral biofilm models have many benefits over in situ research. There are many variables involved in dental calculus formation, such as intra- and inter-individual variation in salivary flow, oral pH, and amylase activity, which can be hard to tease apart in situ. Oral biofilm models provide a controlled environment to explore the effect of selected variables on the growth of calculus and the retention of dietary components in the biofilm, as well as a means to identify how the methods used in archaeology may inadvertently bias the interpretations. This type of research has, so far, been limited, but has the potential to greatly benefit archaeological research on past diet (Radini & Nikita, 2022).
We present an oral biofilm model that can serve as a viable proxy for dental calculus for archaeology-oriented research questions. It is a multispecies biofilm using whole saliva as the inoculate, with a simple multiwell plate setup that is accessible even to smaller lab budgets and those with limited facilities for microbiology work. Here, we used next-generation sequencing and metagenomic classification to characterise the bacterial composition of our model dental calculus and compare it to oral reference samples, including saliva, buccal mucosa, plaque, and modern human dental calculus. This was done to ensure that the model microbiome is predominantly oral and not overgrown by environmental contaminants. We then determined the mineral composition of the model dental calculus using Fourier transform infrared (FTIR) spectroscopy to verify the presence of calculus-specific mineral phases and functional groups, and perform a qualitative comparison with modern and archaeological reference calculus. Overall the model calculus is chemically similar to natural calculus, and has a predominantly oral microbiome. The microbial diversity and richness within the model samples were lower than oral reference samples, suggesting that the model samples do not contain identical species composition and abundances as the natural samples. The mineral composition closely resembles modern and archaeological reference calculus, predominantly comprised of carbonate hydroxyapatite with a similar level of crystallinity and order. As such, the model dental calculus presented here is a viable proxy to natural dental calculus and can be used to explore many of the currently unexplained processes we see in the archaeological material, when working within the limitations of an oral biofilm model.
3.2 Materials and methods
Our biofilm setup consists of whole saliva as the inoculate to approximate natural microbial communities within the human oral cavity, and a 24-well plate to generate multiple replicated conditions in a single experimental run (see Figure 3.1 for an overview of the protocol). The biofilm is grown for 25 days to allow time for growth of larger deposits and mineralisation. Raw potato and wheat starch solutions were added during the biofilm growth to explore the biases involved in their incorporation and extraction from dental calculus. These results are presented in a separate article (Bartholdy & Henry, 2022).
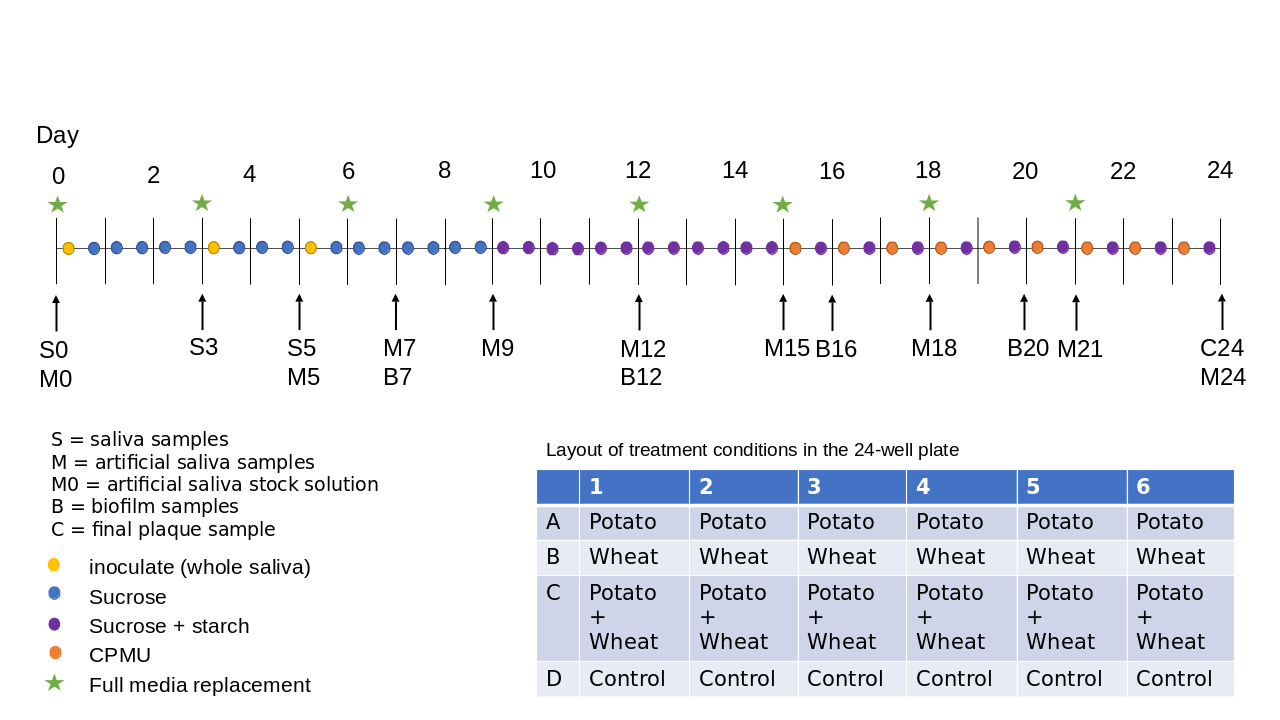
To determine the composition of microbial communities, we sampled the medium from the biofilm wells over the course of the experiment. We sequenced the DNA to identify species that are present in the model, and assess whether these mimic natural oral communities. During a separate experimental run, under the same conditions, we directly sampled the biofilms on multiple days and determined the mineral composition using FTIR, and compared the spectra to those of natural dental calculus, both modern and archaeological. Samples were taken from both controls and starch treatments, but differences between these samples were not explored in this study.
3.2.1 Biofilm growth
We employ a multispecies oral biofilm model following a modified protocol from Sissons and colleagues (1991) and Shellis (1978). The setup comprises a polypropylene 24 deepwell PCR plate (KingFisher 97003510) with a lid containing 24 pegs (substrata), which are autoclaved at 120
The artificial saliva (hereafter referred to as medium) is a modified version of the basal medium mucin (BMM) described by Sissons and colleagues (1991). It is a complex medium containing 2.5 g/l partially purified mucin from porcine stomach (Type III, Sigma M1778), 5 g/l trypticase peptone (Roth 2363.1), 10 g/l proteose peptone (Oxoid LP0085), 5 g/l yeast extract (BD 211921), 2.5 g/l KCl, 0.35 g/l NaCl, 1.8 mmol/l CaCl2, 5.2 mmol/l Na2HPO4 (Sissons et al., 1991), 6.4 mmol/l NaHCO3 (Shellis, 1978), 2.5 mg/l haemin. This is subsequently adjusted to pH 7 with NaOH pellets and stirring, autoclaved (15 min, 120
Fresh whole saliva (WS) for inoculation was provided by a 31-year-old male donor with no history of caries, who abstained from oral hygiene for 24 hours, and no food was consumed two hours prior to donation. No antibiotics were taken up to six months prior to donation. Saliva was stimulated by chewing on parafilm, then filtered through a bleach-sterilised nylon cloth to remove particulates. Substrata were inoculated with 1 ml/well of a two-fold dilution of WS in sterilised 20% glycerine for four hours at 36
On day 9, starch treatments were introduced, replacing sucrose treatments (except for control sample). As with the sucrose treatments, starch treatments occurred twice per day for six minutes, and involved transferring the substrata to a new plate containing a 0.25% (w/v) starch from potato (Roth 9441.1) solution, a 0.25% (w/v) starch from wheat (Sigma S5127) solution, and a 0.5% (w/v) mixture of equal concentrations (w/v) wheat and potato. All starch solutions were created in a 5% (w/v) sucrose solution. Before transferring biofilm samples to the starch treatments, the starch plates were agitated to keep the starches in suspension in the solutions, and during treatments, the rpm was increased to 60. The purpose of starch treatments was to explore the incorporation of starch granules into the model calculus. Starch treatments were initiated on day 9 (Figure 3.1) to avoid starch granule counts being affected by
After 15 days, mineralisation was encouraged with a calcium phosphate monofluorophosphate urea (CPMU) solution containing 20 mmol/l CaCl2, 12 mmol/l NaH2PO4, 5 mmol/l Na2PO3F, 500 mmol/l urea (Pearce & Sissons, 1987; Sissons et al., 1991), and 0.04 g/l MgCl. The substrata were submerged in 1 ml/well CPMU five times daily, every two hours, for six minutes, at 30 rpm. During the mineralisation period, starch treatments were reduced to once per day, two hours after the last CPMU treatment. This cycle was repeated for 10 days until the end of the experiment on day 24 (Figure 3.1). More detailed protocols are available at https://dx.doi.org/10.17504/protocols.io.dm6gpj9rdgzp/v1.
All laboratory work was conducted in sterile conditions under a laminar flow hood to prevent starch and bacterial contamination. Starch-free control samples that were only fed sucrose were included to detect starch contamination.
3.2.2 Metagenomics
A total of 35 samples were taken during the experiment from the donated saliva, artificial saliva, and from the biofilm end-product on day 24 (Table 3.1). DNA extraction was performed at the Max Planck Institute for the Science of Human History (Jena, Germany), using the DNeasy PowerSoil Kit from QIAGEN. C2 inhibitor removal step skipped, going directly to C3 step.
The DNA was sheared to 500bp through sonication with a Covaris M220 Focused-ultrasonicator. Double-stranded libraries were prepared (Aron et al., 2020) and dual indexed (Stahl et al., 2020), with the indexing protocol being adapted for longer DNA fragments. Briefly, the modifications consisted of adding 3 μl of DMSO to the indexing reaction, and extending the amplification cycles to 95
3.2.2.1 Preprocessing
The raw DNA reads were preprocessed using the nf-core/eager, v2.4.4 pipeline (Fellows Yates et al., 2020). The pipeline included adapter removal and read merging using AdapterRemoval, v2.3.2 (Schubert et al., 2016). Merged reads were mapped to the human reference genome (GRCh38) using BWA, v0.7.17-r1188 (Li & Durbin, 2009) (-n 0.01; -l 32), and unmapped reads were extracted using Samtools, v1.12. The final step of the pipeline, metagenomic classification, was conducted in kraken, v2.1.2 (Wood et al., 2019) using the Standard 60GB database (https://genome-idx.s3.amazonaws.com/kraken/k2_standard_20220926.tar.gz).
Environmental reference samples were downloaded directly from ENA and from NCBI using the SRA Toolkit. Oral reference samples were downloaded from the Human Metagenome Project (HMP), and modern calculus samples from Velsko et al. (2017). From the HMP data, only paired reads were processed, singletons were removed. In vitro biofilm model samples from Edlund et al. (2018) were used as a reference. Links to the specific sequences are included in the metadata. Human-filtered reads produced in this study were uploaded to ENA under accession number PRJEB61886.
3.2.2.2 Authentication
Species with lower than 0.001% relative abundance across all samples were removed from the species table. SourceTracker2 (Knights et al., 2011) was used to estimate source composition of the abundance-filtered oral biofilm model samples using a Bayesian framework, and samples falling below 70% oral source were removed from downstream analyses. Well-preserved abundance-filtered samples were compared to oral and environmental controls to detect potential external contamination. The R package decontam v1.22.0 (Davis et al., 2018) was used to identify potential contaminants in the abundance-filtered table using DNA concentrations with a probability threshold of 0.95 and negative controls with a probability threshold of 0.05. Putative contaminant species were filtered out of the OTU tables for all downstream analyses.
3.2.2.3 Community composition
Relative abundances of communities were calculated at the species- and genus-level, as recommended for compositional data (Gloor et al., 2017). Shannon index and Pileou’s evenness index were calculated on species-level OTU tables of all model and oral reference samples using the vegan v2.6.4 R package (Oksanen et al., 2022). Shannon index was calculated for all experimental samples to see if there is an overall loss or gain in diversity and richness across the experiment. Sparse principal component analysis (sPCA) was performed on model biofilm samples to assess differences in microbial composition between samples within the experiment, and a separate sPCA analysis was performed on model calculus and oral reference samples. The sPCA analysis was conducted using the mixOmics v 6.26.0 R package (Rohart et al., 2017).
The core microbiome was calculated by taking the mean genus-level relative abundance within each sample type for model calculus, modern reference calculus, sub- and supragingival plaque. Genera present at lower than 5% relative abundance were grouped into the category ‘other’. Information on the oxygen tolerance of bacterial species was downloaded from BacDive (Reimer et al., 2022) and all variations of the major categories anaerobe, facultative anaerobe, and aerobe were combined into the appropriate major category. At the time of writing, 55.7% species were missing aerotolerance values. This was mitigated by aggregating genus-level tolerances to species with missing values, and may have some errors (although unlikely to make any significant difference).
3.2.2.4 Differential abundance
Differential abundance of species was calculated using the Analysis of Compositions of Microbiomes with Bias Correction (ANCOM-BC) method from the ANCOMBC R package v2.4.0 (Lin & Peddada, 2020), with a species-level OTU table as input. Results are presented as the log fold change of species between paired sample types with 95% confidence intervals. P-values are adjusted using the false discovery rate (FDR) method. Samples are grouped by sample type (i.e. saliva, plaque, modern calculus, model calculus). To supplement the sPCA analyses, we visualised the log-fold change of the top 30 species in each of principal components 1 and 2, allowing us to see which species are enriched in the different samples and causing clustering in the sPCA.
3.2.3 FTIR
To determine the mineral composition and level of crystallisation of the model dental calculus samples, we used Fourier Transform Infrared (FTIR) spectroscopy. We compared the spectra of model dental calculus with spectra of archaeological and modern dental calculus and used a built-in Omnic search library for mineral identification (Mentzer et al., 2014; Weiner, 2010b). The archaeological dental calculus was sampled from an isolated permanent tooth from Middenbeemster, a rural, 19th century Dutch site (Lemmers et al., 2013). Samples were analysed at the Laboratory for Sedimentary Archaeology, Haifa University. The analysis was conducted with a Thermo Scientific Nicolet is5 spectrometer in transmission, at 4 cm
Analysis was conducted on 26 model calculus samples from days 7, 12, 16, 20, and 24 (Table 3.2). Some samples from the same sampling day had to be combined to provide enough material for analysis. Samples analysed with FTIR were grown during a separate experimental run from the samples sequenced for DNA, but following the same setup and protocol (as described above). Samples were analysed following the method presented in Asscher, Regev, et al. (2011) and Asscher, Weiner, et al. (2011). A few
3.2.4 Statistics
Statistical analysis was conducted in R version 4.3.2 (2023-10-31) (Eye Holes) (R Core Team, 2020). Data cleaning and wrangling performed with packages from tidyverse (Wickham et al., 2019). Plots were created using ggplot2 v3.4.4 (Wickham, 2016).
3.3 Results
3.3.1 Metagenomic analysis
3.3.1.1 Sample authentication
To determine the extent of contamination in our samples, we performed a source-tracking analysis using SourceTracker2 (Knights et al., 2011). Results suggest that the majority of taxa across samples have an oral microbial signature, and therefore our samples are minimally affected by external contamination (Figure S1). We compared SourceTracker2 results to a database of oral taxa from the cuperdec v1.1.0 R package (Fellows Yates et al., 2021) to prevent removal of samples where oral taxa were assigned to a non-oral source (Figure S2), as some taxa with a signature from multiple sources are often classified as “Unknown” (Velsko et al., 2019). We included several oral sources, which may increase the risk of this occurring. Samples containing a large proportion (>70%) of environmental contamination were removed. The removed samples were predominantly medium samples from later in the experiment, and a few model calculus samples. After contaminated samples were removed, suspected contaminant-species were removed from the remaining samples using the decontam R package (Davis et al., 2018). After contamination removal, samples consisted of between 88 and 284 species with a mean of 182.
3.3.1.2 Decrease in community diversity across experiment
To monitor the development of microbial communities over the course of the experiment, we used the Shannon Index to assess the species diversity and richness at various stages of our protocol. Samples were grouped into sampling categories due to low sample sizes on sampling days (inoc = days 0, 3, 5; treatm = days 7, 9, 12, 15; model = day 24). There was a slight decrease in mean Shannon Index between inoculation and treatment samples, followed by a slight increase to model calculus samples, as well as a decrease in variance within sample types. The Pielou Evenness Index showed a similar pattern while the number of species increased between the treatment period and the final model calculus (Figure 3.2).
3.3.1.3 Medium and model calculus samples are distinct from the inoculate
We next examined whether there is a change in the species composition over time in our samples by assessing the beta-diversity in a PCA. The species profiles of the saliva inoculate used in our experiment were distinct from both medium and model calculus samples. Most of the separation of saliva from model calculus is on PC1 of the sPCA, where most of the positive sample loadings are driven by anaerobic species (model calculus), especially Selenomonas spp, and negative loadings are predominantly facultative anaerobes and some aerobes, such as Rothia and Neisseria spp (saliva). Medium and saliva are separated mostly on PC2, with medium samples located between saliva and model calculus samples. Model calculus samples also cluster separately from the medium samples on PC2, with some overlap between the more mature medium samples and model calculus. Most of the negative loadings separating saliva and model calculus from medium samples are dominated by Actinomyces spp., while positive species loadings are more diverse, and seemingly unrelated to aerotolerance (Figure 3.3).
We determined whether there are species that are differentially abundant between our sample types using the ANCOMBC R package (Lin & Peddada, 2020), giving us an idea of how the biofilm develops under our experimental conditions. Species enriched in saliva compared to model calculus are largely aerobic or facultatively anaerobic, while species enriched in model calculus compared to saliva are mainly anaerobes. The differences between saliva and calculus are more pronounced than between medium and model calculus, which is expected (Figure 3.4).
3.3.1.4 Lower diversity in artificial samples than oral references
We used the Shannon Index to compare alpha-diversity in our model to oral reference samples. The mean Shannon Index of model samples—medium, model calculus, reference in vitro biofilm were consistently lower than the means of oral reference samples—mucosa, modern reference dental calculus, saliva, and subgingival and subgingival plaque. The Pielou species evenness index has a similar distribution, although the comparative biofilm samples have a higher mean than biofilm samples from this study. Saliva inoculate samples from this study have a lower mean Shannon index than reference samples, which may have contributed to the lower alpha-diversity in model samples compared to reference samples. The number of species follows the same trend.
3.3.1.5 Model calculus is distinct from dental calculus and other oral samples
We calculated the mean relative abundances of the genera in each sample to compare the core genera of model calculus with oral reference samples. The most common genera (>5% relative abundance) are shown in Figure 3.6. The main overlap between the model calculus and oral reference samples is the high relative abundance of Streptococcus. Model calculus consists mostly of Enterococcus and Veillonella spp., despite both having low abundance in donor saliva. Enterococcus are also known environmental contaminants, and we cannot exclude environmental contamination as a possible source for these species in our model. Oral reference samples have a more balanced composition, as they are also represented by fastidious early-coloniser species like Capnocytophaga and Neisseria spp., which require an environment with at least 5% carbon dioxide to thrive (Tønjum & van Putten, 2017).
To directly compare the beta-diversity of our model calculus with oral reference samples, including modern dental calculus, we used an sPCA including only our model calculus and reference samples. Model calculus samples are distinct from both the oral reference samples and the biofilm model reference samples. They are separated from oral reference samples mainly on PC1, and from biofilm model reference samples (and, to some extent, oral samples) on PC2. The highest negative contributions are a mix of all types of aerotolerance, while the positive contributions are mostly (facultative) anaerobes, with Enterococcus spp. as the top three positive contributors to PC1. Top negative contributors are Capnocytophaga spp as well as the aerobes Corynebacterium matruchotii and Rothia dentocariosa. The top positive contributors to PC2 are all anaerobes, mainly from the genus Selenomonas. Top negative contributors to PC2 are a mix of aerotolerances, with many Streptococcus spp (Figure 3.7).
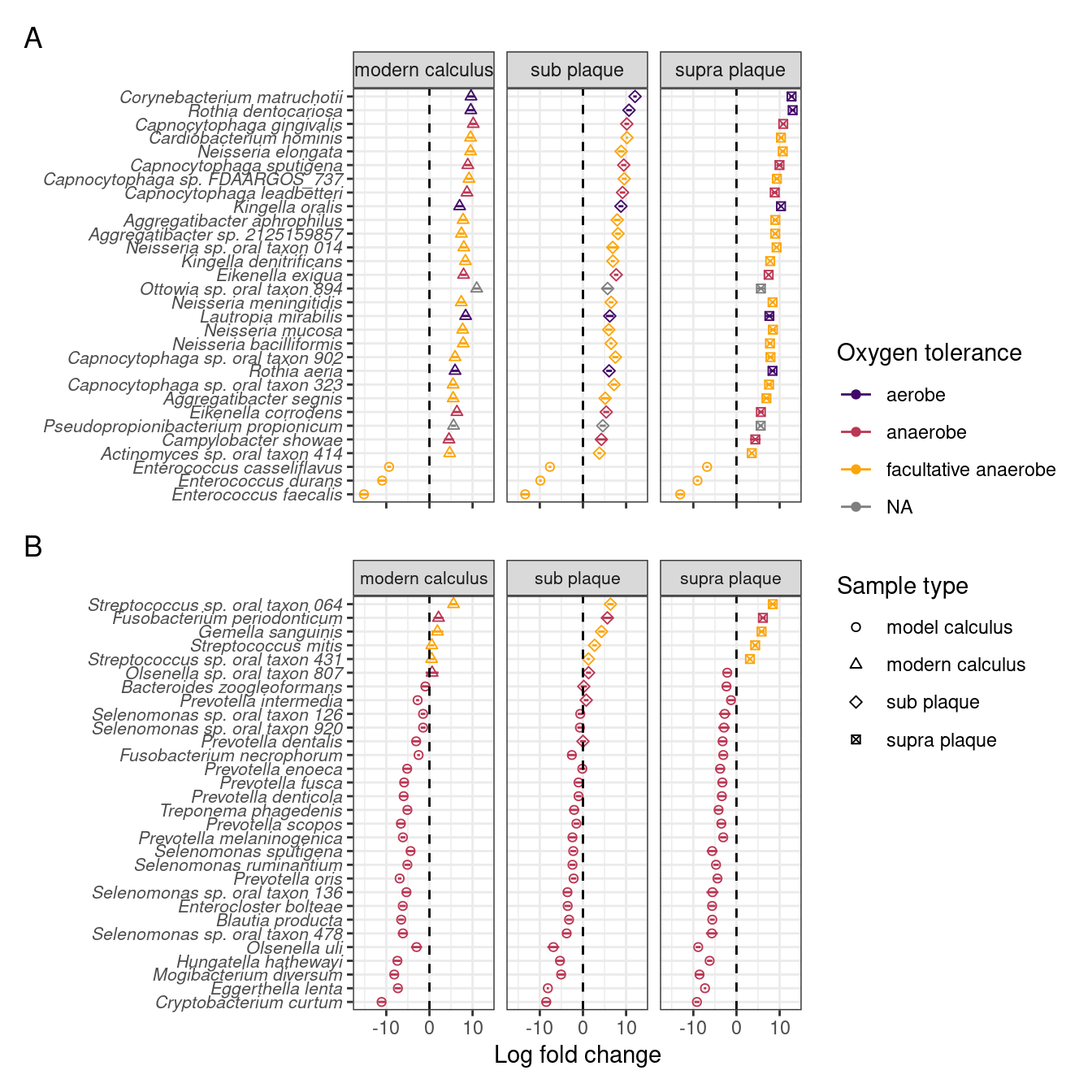
To investigate which species are enriched in different sample types, and compare the final product of our model with naturally occurring plaque and calculus samples, we performed differential abundance analysis on our model calculus samples, modern dental calculus, and sub- and supragingival plaque. Based on the differential abundance analysis the main differences between model calculus and oral reference samples, when looking at the top 30 contributors to PC1, are that the oral reference samples are enriched with species with a diverse oxygen tolerance from a wide range of genera, while the model calculus is enriched with Enterococcus spp. The largest differences occur in Corynebacterium matruchotii, Rothia dentocariosa, and Capnocytophaga gingivalis (Figure 3.8A). This is echoed when looking at the top 30 contributors to PC2, where most of the species are enriched in model calculus, all of which are anaerobes, and the largest differences occurring in Cryptobacterium curtum, Eggerthella lenta, and Mogibacterium diversum (Figure 3.8B).
3.3.2 Samples show an increased mineralisation over the course of the experiment
To determine whether the model dental calculus is comparable to natural dental calculus, both modern and archaeological dental calculus were analysed with FTIR spectroscopy to ascertain their composition.
It is evident that between days 7 and 24 there is a decrease of the protein components and increase of the inorganic mineral carbonate hydroxyapatite. The model calculus samples from the end of the experiment are similar to both the modern and archaeological reference samples. The main difference is a lower organic component in reference samples seen as a reduced amide I peak at around 1637 compared to the carbonate peak at around 1420, and an absence of amide II and III. Further, there is a reduction in CH3 bands at 3000-2900 cm
Sample spectra from days 7 and 12 are characterised by a high content of proteins as evident by the strong amide I absorbance band at 1650, a less pronounced amide II band at 1545 cm
In spectra from days 16 to 24, the ratio of amides to PO4 has shifted, with the main peak shifting to the PO4 v3 absorbance band at 1039–1040 cm
3.3.3 Model calculus has a similar mineral composition to natural calculus
Archaeological and modern reference spectra are largely indistinguishable and consist of a broad O–H absorbance band (3400 cm
3.3.4 Samples show similar crystallinity and order to reference calculus
We determined the level of crystallinity and order of the carbonate hydroxyapatite in our samples as an indication for its maturity by using the grinding curves method presented by Asscher, Regev, et al. (2011) and Asscher, Weiner, et al. (2011).
Samples were compared to published trendlines for archaeological and modern enamel (Asscher, Regev, et al., 2011). We see no appreciable differences between days 16, 20, and 24. The archaeological dental calculus shows a slightly increased slope compared to model calculus from the three sampling days used in the grind curve (Figure 3.10), possibly indicating larger crystal size due to more complete crystalisation. The steeper slope of enamel samples is consistent with a more ordered structure in enamel compared to dental calculus.
3.4 Discussion
In this study we present a calcifying oral biofilm model to produce artificial dental calculus. Our proposed use of the model is to address a variety of research questions related to dietary information extracted from dental calculus, in both modern and archaeological samples. For that to be feasible, the model needs to serve as a viable proxy to dental calculus grown under natural conditions, i.e., in the human oral cavity. It needs, as much as possible, to mimic the diversity and complexity of the natural oral microbiome, while also offering control over factors such as dietary input, growth conditions, and replicability within and between experiments. Here, we assessed the viability of our model as a proxy for dental calculus using metagenomic classification and FTIR analysis to explore the bacterial and mineral composition, and compare with oral reference samples.
3.4.1 Microbiome
Model calculus has lower species diversity than inocula saliva and oral reference samples, which is a common limitation in biofilm models (Bjarnsholt et al., 2013; Edlund et al., 2013). The donated saliva for the experiment had a lower diversity than the reference saliva samples, and may have contributed to a lower diversity in experimental samples. Consequently, there is also a lower diversity and richness when compared to other modern oral reference samples, including oral mucosa, saliva, plaque, and calculus. Samples of the medium from early in the experiment have similar species profiles to the donated saliva, but gradually diverge over the course of the experiment. This may be caused by experimental setup not sufficiently mimicking the oral environment, allowing species to thrive that do not normally thrive in the natural oral environment.
Oral reference samples have a relative abundance of streptococci similar to our model, but a more diverse representation from other genera and an overall higher species diversity and richness than our model. Reference samples also had a more diverse aerotolerance profile than our model, which primarily consisted of (faculatative) anaerobes. Species within predominantly aerobic genera, are deficient in the model, suggesting a shift from a largely aerotolerant profile to an anaerobic profile during the experiment. While our model is not set up as an anaerobic system, the anaerobes seem to have outcompeted aerobes and, to some extent, facultative anaerobes. This is likely a result of communities of bacteria within the biofilm creating favourable microenvironments facilitated by the protective properties of the biofilm matrix (Edlund et al., 2018; Flemming et al., 2016).
Overall, the majority of model calculus samples contained a distinctly oral signature, providing a promising starting point for the use of the model as a viable proxy to dental calculus. The main differences between model and oral reference samples may be due to human variation, as there can be large differences in the oral microbiome of two individuals at the species level due to variations in age, sex, and other demographic factors, as well as how and when saliva samples were collected (Burcham et al., 2020; Nearing et al., 2020). Whether or not distinct microbial profiles, and the extracellular matrix they produce, will affect the retention of dietary particles in plaque remains to be seen, but is an important question to address in the future.
3.4.2 Mineralisation
FTIR analysis allowed us to address the mineralisation process of the model, which showed an increasing mineral composition over the course of the experiment. As the model biofilm matured, the predominantly organic content of early samples was replaced by inorganic content in the form of carbonated hydroxyapatite, consistent with a shift from a high presence of bacterial cells in a matrix of extracellular polysaccharides (Jain et al., 2013; Sutherland, 2001; Zhang et al., 1998) to a predominantly mineral content.
The model calculus samples resemble both the modern reference calculus and the archaeological calculus in mineral composition and crystallinity. The steeper slope in the grind curve plots of the archaeological sample suggests that the crystals in archaeological samples are larger, and hence more ordered than in model calculus. A possible explanation is that the inorganic crystals within archaeological calculus have had more time to grow into the space left by degraded organic matter (Weiner, 2010a); however, we only analysed one archaeological sample and cannot definitively address this. The short duration of model calculus growth may also have affected the results, compared to the longer-term growth and mineralisation of natural calculus. The constant disruptions in growth of in vivo dental plaque/calculus, due to oral hygiene and other external pressures on biofilm growth, may lead to multiple stages of calcium phosphates, whereas our model has more stable growth conditions.
One of the most well-known biomineralisers, Corynebacterium matruchotii (Ennever et al., 1978; Takazoe et al., 1970), exhibited a lower abundance in our model calculus compared to modern reference calculus. However, the mineral composition of the end results were similar, reinforcing the idea that, under the right circumstances, biofilms with a range of microbial profiles can facilitate mineralisation (Moorer et al., 1993). Bacteria and their ability to secrete an extracellular matrix are integral in the formation of dental calculus, and inevitably serve as part of the structure that dental calculus is built upon (Rohanizadeh & LeGeros, 2005), while the exact species composition of the biofilm communities may be less important.
3.4.3 Replicability
Model calculus displayed similar species diversity and microbial profiles across all samples, indicating a high level of replicability between samples in the experimental run. It remains to be seen whether the replicability within the experiment also scales up to between-experiment replicability in our model, though others have already shown that replicability in long-term models is possible when using the same inocula (Velsko & Shaddox, 2018). The variation in mineral composition in our model was initially high, but samples from day 24 were largely similar in composition as observed in the FTIR spectra. The use of a simple multiwell plate setup allows us to submit many samples to the same conditions, increasing replicability between samples (Exterkate et al., 2010).
3.4.4 Limitations
While our in vitro model calculus system provides reproducible and consistent artificial dental calculus for archaeological research, as demonstrated by the species composition and the mineralisation properties, we recognise the model has several limitations. Our single-donor approach may have affected the diversity of the model. The donated saliva from our study had a lower mean Shannon Index than other saliva samples. The lower diversity may be caused by only using one donor instead of pooling saliva from multiple individuals. However, having a single inoculum donor allows us to maintain the integrity of a native oral microbiome which may be lost when samples are pooled (Edlund et al., 2013). It is also possible that the diversity was affected by the collection and storage methods we used. This has been shown to have minimal effect on microbial profiles at the genus level (Lim et al., 2017), but some effect on beta diversity calculations (Omori et al., 2021).
Some samples were grown with starch-sucrose solutions as nutrients, while controls were grown with sucrose only. Due to the financial cost, we did not sequence enough samples of each nutrient treatment to assess the influence of starch on the microbial community or mineral composition. Biofilms were grown in a standard shaking bacterial growth incubator, rather than an incubator specific to cell cultures. The lack of complex environmental control may cause the model to deviate from its natural growth over the 25 days that the experiment is run, due to a lack of precise control over conditions such as pH and salivary flow rates.
There is also the possibility that contamination was introduced into the model during the experiment. While the CPMU solution was prepared under sterile conditions, the solution itself was not autoclaved or filter-sterilised. In the species composition metagenomic analysis, all medium samples collected after the introduction of CPMU on day 14 were removed by the authentication step because the majority of species appeared to derive from environmental sources indicating external contamination. Going forward we recommend filter-sterilising solutions that are not autoclaved.
To avoid disturbing the growth and development of our biofilm, we took samples of media from the bottom of the wells after three days without full media replacement, careful not to disturb other plate-bound biofilms. The samples may therefore not fully reflect the composition of the biofilm itself. Going forward we recommend sampling from the actual biofilm, as this is the sample type under investigation.
3.4.5 Future work
Further protocol optimisation will also be necessary to address some of the limitations of our current model, such as reducing the frequency of medium replacement (currently every three days) to help promote the growth of slow-growing fastidious organisms and limit generalists such as enterococci, and supplementing it with serum to provide additional nutrients and biofilm stability (Ammann et al., 2012; Tian et al., 2010). More infrequent medium replacement would facilitate slow-growing bacteria in establishing their metabolic relationships, allowing the byproducts of some species to become abundant enough for others that depend on these to grow (Marsh, 2005).
Our goals for additional validation measures involve functional profiles of bacteria, to see if metabolic behaviour of bacteria is consistent with in vivo conditions, and whether this is affected by the presence/absence of amylase and starch treatments. The absence of host salivary
The model can also be used to explore limitations and biases of methods used to reconstruct past dietary patterns from dental calculus. To this end, sucrose and raw starch treatments can be replaced with other dietary components of interest, such as cooked starches, whole plant extracts, and various proteins.
3.5 Conclusions
The bacterial profile of our model calculus is not an exact match to the natural modern or archaeological reference calculus, but species richness and diversity falls within a similar range as the reference in vitro model, and the core genera are predominantly oral. Our model calculus had a distinct microbial profile from modern reference calculus, but a similar mineral composition to modern and archaeological reference calculus, consisting of carbonate hydroxyapatite and similar levels of crystallinity and order, with a slightly higher organic phase.
Our model has many potential benefits within archaeological research, especially since the setup does not require highly specialised equipment, making it accessible to many labs within the archaeological sciences. It can be used to test many fundamental aspects of the process of incorporation, retention, and subsequent extraction of various dietary components from archaeological dental calculus. Using an oral biofilm model in a controlled environment with known dietary input, we can learn more about how different methods of food processing in the past may affect results of dental calculus analyses, and how the methods we use may further distort this picture. Our method can be used to test methods (e.g. DNA, proteomics, etc.), decontamination protocols, as well as training on these methods and protocols without depleting limited archaeological resources. The purpose of our model is not to replace studies conducted on archaeological and natural dental calculus, but rather to balance limitations of each method and serve as a complementary approach to expand our toolkit.